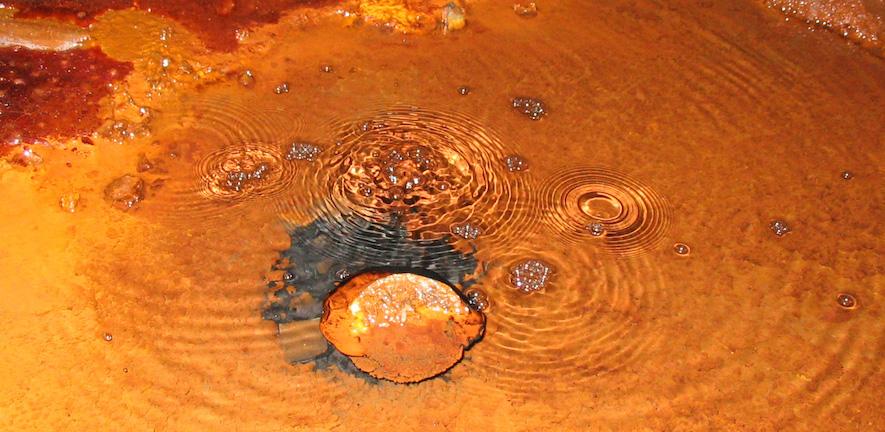
Submitted by Administrator on Wed, 15/09/2021 - 09:09
Prebiotic chemistry deals with the synthesis and interaction of those organic compounds which might have been present before life began. So, what are sufficient conditions for the synthesis of the organic molecules constituting the building blocks of life as we know it?
Dr Paul Rimmer of Cambridge’s Department of Earth Sciences who is also affiliated with Cambridge’s Cavendish Laboratory and MRC Laboratory of Molecular Biology brought together three Earth scientists, Professor Alexis S. Templeton (University of Colorado), Professor Nick Tosca (University of Cambridge) and Professor Barbara Sherwood Lollar (University of Toronto), to discuss mechanisms involving the emergence of such chemistry under different conditions billions of years ago on Earth and potentially on other planets and moons.
The typical approach scientists take when trying to unravel ways in which chemical compounds, such as hydrogen cyanide, cyanoacetylene, or formaldehyde — as suggested by Rimmer — might have emerged, involves three key aspects: investigate whether places on Earth might have conditions that are like the ones present billions of years ago, try reproducing these mechanisms in a lab, and lastly come up with simulations. This culminates in the development of models that might explain plausible ways in which these compounds might have formed as well as potentially clarify what to look for outside Earth when trying to identify planets that might bear signs of life or biosignatures.
Rimmer opened the discussion with an introduction on the importance of hydrogen in prebiotic chemistry. Excess of hydrogen on Earth might have originated from a variety of processes. One process involves giant comet impacts and the iron they carry with them, followed by the reaction of iron with water and the release of hydrogen. Hydrogen can lead to reducing conditions essential for prebiotic chemistry. Also, hydrogen is a source of food effectively for early life.
The panelists presented various geochemical sources of H2.
Templeton, Professor of Geobiology and Geochemistry at the University of Colorado Boulder, spoke about “H2 generation and releasing the reducing power of iron silicate rocks”.
“A lot of the time in the interests of discussions of serpentinization, there's a focus on the fact that to maximize the yield of molecular hydrogen that's generated, a lot of models, depending on how they're designed, will predict that you would get a maximum amount of hydrogen somewhere often around 300°C”, said Templeton. Later in her talk, Templeton showed that H2 is produced in prebiotically and biologically relevant quantities at much lower temperatures than 300°C, even at near room temperature. The yield of H2 is closely related to the amount of magnetite formed and olivine is what we often think of in a planetary context as being the initial source term for hydrogen generation.
Templeton is interested in investigating the reactivity of these minerals not only in relation to H2 production, but also other reduced species, including ammonia and cyanide. “I think that as we keep improving this understanding of the mechanisms that control hydrogen generation, we're also going to effectively move into a better understanding of the potential for which transformations are feasible with nitrogen, phosphorus, carbon and sulfur.”, Templeton concluded.
Tosca, Professor of Mineralogy and Petrology at the University of Cambridge, spoke about “Fluid mixing and low-temperature H2 production on early Mars (and Earth?)”.
Tosca commented on his involvement in recent Mars missions: “Our interest in hydrogen started with the Mars Science Laboratory rover, which ended up landing on the surface of Mars in 2012, and when that rover started analyzing a succession of mudstones and sandstones preserved on the surface of Mars.”
Mars exploration is fascinating because its surface preserves an extensive sedimentary record, whereas on Earth, most of these rocks have long ago been obliterated, so “if one can get a rover to the surface of Mars and be wise in picking an outcrop or a succession that can constrain paleoenvironments, then you can come up with a series of observational constraints and what early planetary environments might have been like”, said Tosca.
Tosca mentioned that on Mars there’s plenty of magnetite and part of his studies involved trying to replicate the mechanisms that might have led to the formation of this mineral on Mars. Referring to one of his experiments he said “…over a couple of days or so, we believe that the green rust is transforming to magnetite, not through a solid-state transformation, but a dissolution reprecipitation transformation, simply due to its thermodynamic metastability with respect to magnetite. This is a really interesting mechanism and has the potential to produce rather large quantities of hydrogen.”
Another known source of abiotic hydrogen on Earth is radiolysis in deep waters. This was introduced by Sherwood Lollar, University Professor in Earth Sciences at the University of Toronto.
“Although there’s been a lot of destruction, there’s still a lot of billion-year-old real estate on this planet”, commented Sherwood Lollar, who’s spent 30 years of her life exploring the hydrogeology and microbiology of environments several kilometers deep in the ground in places like South Africa, Finland, Australia, and Canada.
In some of the water reservoirs that fill up these fractures one can observe “active exsolution of a gas phase that can be up to 50% H2”. While excess H2 has commonly been attributed to serpentinization in ultramafic rocks, in the 90s, Sherwood Lollar unexpectedly discovered analogous excess H2 in places like the Witwatersrand Basin (South Africa) which lacks ultramafic rocks. She attributed the excess H2 to radiolysis, a phenomenon first described by Marie Curie in her 1910 Treatise on Radioactivity. Radiolysis is the dissociation of molecules by ionizing radiation, such as α, β and γ particles, which can be emitted by ubiquitous radioactive materials like uranium, thorium or radium.
“When you have this radiolytic decomposition of water, you produce hydrogen, but all of these reactive oxides then can also attack solid materials, in particular, sulphide minerals, pyrite, pyrrhotite”, added Sherwood Lollar. This has strong implications for the emergence of non-photosynthetic life because radiolysis provides “a coherent long-term mechanism capable of supplying both the electron donor and the electron acceptor, and thereby an answer to how it is you could sustain microbiology in such isolated systems”.
From observations conducted at the Kidd Creek Mine in Canada and studies on nuclear waste disposal, Sherwood Lollar discovered that radiolysis can also produce a series of organic acids, particularly acetate, formate, and oxalate, in the presence of calcium carbonate. These are fundamental molecules for energy metabolism in living cells.
“Radiolytic chemistry can not only give us insight into hydrogen, sulfur and carbon, but may indeed be key to providing a mechanism for sustaining deep subsurface microbes in the absence of interaction with the surface photosphere. In the context of planetary habitability, this may provide some models to think about in terms of how chemoautotrophic life might be sustained on planets when perhaps photosynthesis never evolved”, said Sherwood Lollar.
A recent paper published in Scientific Reports, in fact, suggests that microbial habitability of icy moons (like Enceladus and Europa) could be sustained by radioactive sources, such as uranium, thorium, and potassium.
Should radioactive materials be added to the list of prebiosignatures?
During the discussion, Dr Ziwei Liu from the MRC Laboratory of Molecular Biology raised several interesting questions about these different sources of organic molecules, and discussed new work that he leads within the Sutherland Group. Dr Liu discovered that exposing sulfites and carbon dioxide to ultraviolet light leads to the synthesis of several of the organic molecules required for the Krebs Cycle, such as malate, succinate, and citrate.